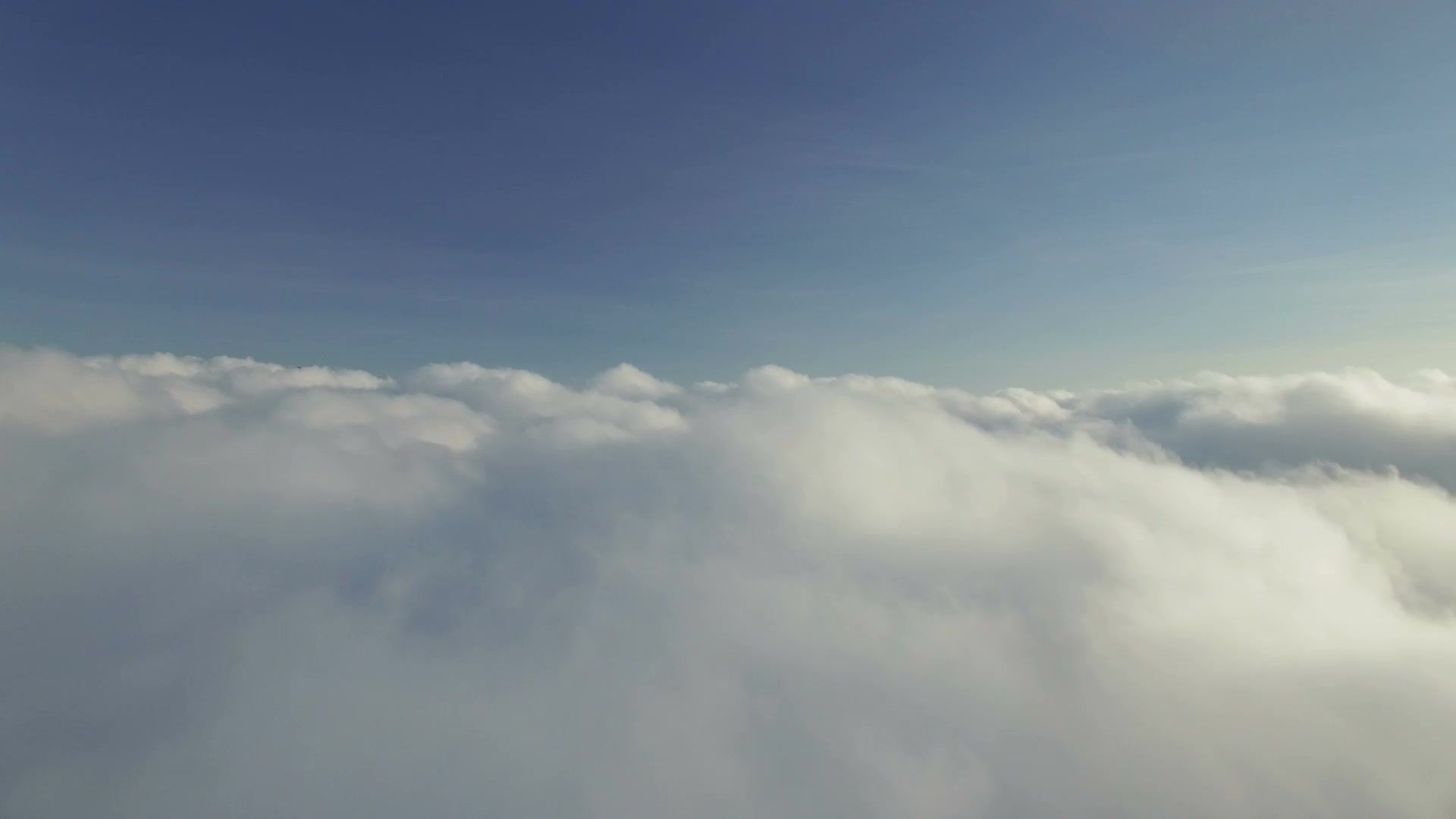
Aerothermochemistry
We are currently involved in collaborative projects with Rodney Bowersox of the Aerospace Engineering Department as part of the National Aerothermochemistry and Hypersonics Laboratory (NAL) (formerly as part of the National Center for Hypersonic Laminar-Turbulent Transition Research) at Texas A&M University. Our research focuses on optical characterization of high speed (hypersonic) flows; measurement of freestream turbulence, measurement of internal energy distributions and relaxation of non-thermal equilibrium (NTE) distributions, surface ablation and reactivity, and laser induced NTE driven turbulence. These measurements require the application of state-of-the art laser diagnostic techniques such as coherent anti-Stokes Raman spectroscopy (CARS), spontaneous, Raman, and two-line planar laser induced fluorescence (PLIF).
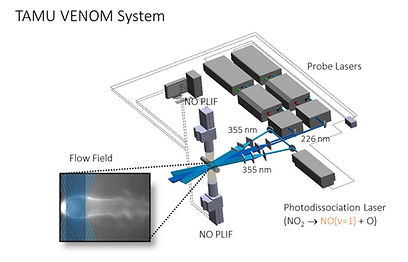
Novel Laser Diagnostics
We have recently developed and characterized a laser based diagnostic technique to characterize unsteady NTE flows. Molecular tagging velocimetry (MTV) is a powerful alternative to the more common particle imaging velocimetry (PIV) technique. Traditional PIV often suffers from
nonuniform particle seeding, inaccuracies in tracking velocities across strong shocks, and a prohibitive degree of light scattering near
surfaces. The vibrationally excited NO Monitoring (VENOM) technique is an extension of two-component MTV using NO(v=1) generated from the photodissociation of seeded NO2, where the two sequential fluorescence images are obtained probing two different rotational states to provide both
velocity and temperature maps. Velocity and internal state distributions represent critical parameters in the characterization of complex
and reacting flowfields. To our knowledge there is currently no single optical diagnostic technique capable of simultaneous measurements of these properties in a plane.
Two ‘read’ lasers pulses, following photolytic production of NO, excite different NO rotational states with a time delay to allow displacement. Differences between the fluorescence images provides the two-component velocity field. Following dewarping, the ratio of images can be used to determine the rotational/translational temperature of the flow. Shown to the right is an image pair of an axisymmetric underexpanded jet obtained using the VENOM technique. The NO rotational states have been chosen to provide accurate two-line temperature determination for this specific flow. Since the initial image, in practice is time delayed from the well- characterized ‘write’ grid, it is possible to extract acceleration from such data. The advantage of using NO2 photolysis in an MTV experiment resides in being able to overcome the short fluorescence lifetime of NO in high quenching environments and in having the possibility of probing the vibrationally excited photofragment to discriminate from environments with background NO.

Thermal disturbance of the flow, however, remains a potential problem when high-photolysis laser powers are employed. An alternative method to perform these measurements, retaining the advantages of using vibrationally excited NO without the
significant thermal disturbance of the photolysis approach, consists in performing tagging of an NO-containing flow by electronic excitation of NO. Electronic excitation of NO in the gamma bands is followed by the formation of vibrationally excited NO by spontaneous emission and collisional quenching. Spontaneous emission from the A2+ state of NO favors low-v” states according to Franck-Condon transition probabilities, peaking at v”=1 with a 28% yield. This results in enough population that can be subsequently probed by additional electronic excitation probes to track flow motion. This approach is named “invisible ink” and is shown below to the left. Demonstration of the technique in an underexpanded jet is shown below
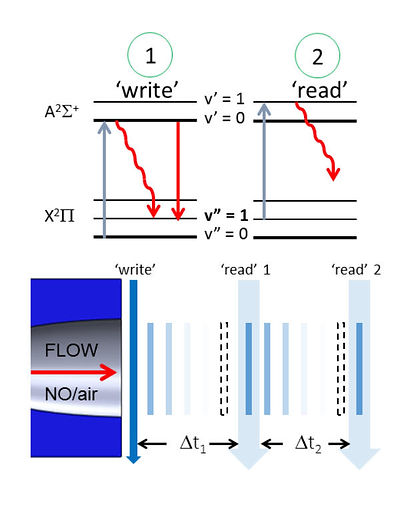
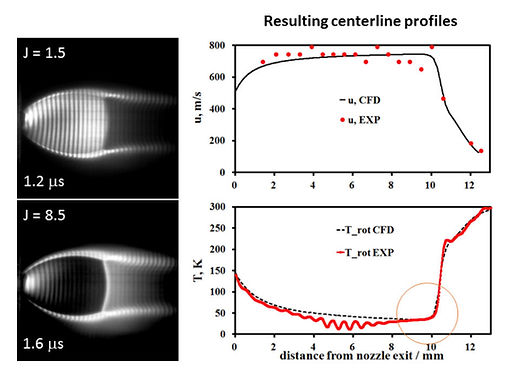
Recently we have demonstrated the viability of the simultaneous measurement of three components of velocity and planar temperature across an oblique shock wave by the excitation of two different transitions in the A-X (1, 1) band of vibrationally excited NO formed by spontaneous emission and collisional quenching from initially excited NO (A 2Σ+). Three- component velocity determinations were derived from two dimensional molecular tagging velocity measurements employing sequential fluorescence images pair obtained simultaneously by two cameras in stereoscopic configuration. The resulting velocity component plots are shown below where the streamwise velocity component in the pre-shock area results in 760±14 m/s within 2.8% deviation from the predicted value of 739 m/s, which is comparable to our previous reports of streamwise motion determinations under similar conditions. The post-shock streamwise velocity component decreases to 672±20 m/s, which is consistent with the calculated value of 657±9 m/s, based on a wedge deflection angle of 16 ± 1 degrees. The out-of-plane velocity measurement in the post-shock resulted in a value of 169 ± 8 m/s, within 10% deviation from the expected value of 188±10 m/s. The out-of-plane velocity component before the shock wave is consistent with the expected value of 0 m/s, while the radial velocity component is negligible as predicted in both pre-shock and post- shock areas. The stereoscopic VENOM measurements resulted in an average temperature of 54 K in the pre-shock area, 6 K higher than the temperature obtained from NO PLIF and 20 K higher than the calculated temperature based on the nozzle Mach number. However, the temperature measured downstream from the shock resulted in a value of 69±2 K, showing better agreement with the predicted temperature of 72±4 K.


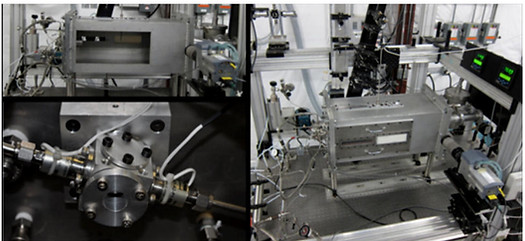
Repetitively Pulsed Hypersonic Test Chamber
We have recently constructed and characterized a repetitively pulsed hypersonic test facility which was developed to enable laser diagnostic development and exploratory experiments (see below). We have demonstrated pulsed (5-15 ms pulse length) uniform hypersonic flows at M=2.9, M=3.8, M=4.6 and M=6.2 for extended operation (>8 hours). The pulsed flow output can be easily synchronized with laser and camera systems using conventional digital delay generators. The system is particularly well suited to longtime operation often required for diagnostic development and/or optimization, and for the use of toxic chemical tracers which can be cryogenically trapped at the modest flows employed. The pulse-to-pulse total pressure repeatability was within 1%. The measured exit flow velocity and temperature, measured using NO MTV/PLIF, were uniform to within 1.5% and 3.7% for the M=4.6 and M=6.2 nozzles, respectively.